What Are the Key Capacity Requirements for LiFePO4 Batteries in Different Applications?
LiFePO4 batteries require tailored capacity planning based on application-specific energy demands, discharge rates, and environmental conditions. Key factors include load profiles, cycle life expectations, temperature ranges, and space constraints. For example, solar storage prioritizes deep-cycle longevity, while EVs focus on high energy density and rapid charging. Proper capacity calculation ensures optimal performance, safety, and cost-efficiency across industries.
What factors should you consider when choosing LiFePO4 batteries?
How Do LiFePO4 Batteries Compare to Other Chemistries in Capacity?
LiFePO4 batteries offer lower energy density (120–160 Wh/kg) than NMC or LCO cells but excel in thermal stability, cycle life (2,000–5,000 cycles), and flat voltage curves. Their capacity remains consistent under high discharge rates, making them ideal for applications requiring steady power output, such as UPS systems or marine electronics, where safety and longevity outweigh volumetric energy constraints.
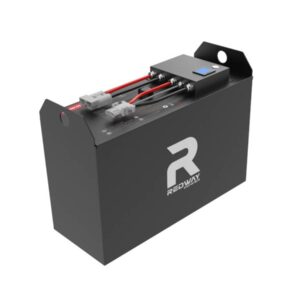
What Formulas Calculate LiFePO4 Capacity for Specific Applications?
Capacity (Ah) = (Load Power (W) × Runtime (h)) / Battery Voltage (V). Adjust for depth of discharge (DoD) and efficiency losses (typically 15–20%). Example: A 1,000W solar load requiring 8 hours at 24V with 80% DoD needs (1,000×8)/(24×0.8) = 416Ah. Real-world derating for temperature and aging adds 10–25% buffer.
Which Environmental Factors Impact LiFePO4 Capacity Most?
Temperature extremes reduce usable capacity—LiFePO4 operates best at 15–35°C. Below 0°C, capacity drops 20–30%; above 45°C, cycle life degrades. Humidity accelerates corrosion in terminals, while vibration in automotive/marine applications can loosen connections, causing uneven cell discharge. Altitude has negligible direct impact but correlates with thermal management challenges.
Which LiFePO4 car starter battery is best for your vehicle?
Temperature Range | Capacity Retention | Recommended Mitigation |
---|---|---|
-20°C to 0°C | 60-75% | Heated battery blankets |
15°C to 35°C | 100% | Natural convection cooling |
45°C to 60°C | 85-90% | Active liquid cooling |
Recent field studies show that coastal installations require special attention to saltwater exposure. Marine-grade LiFePO4 batteries with IP67 ratings demonstrate 12% better capacity retention after 5 years compared to standard models. In desert environments, daily temperature swings exceeding 30°C can accelerate cell aging—insulated battery compartments with phase-change materials help maintain stable thermal conditions.
How Does Temperature Management Optimize LiFePO4 Capacity?
Active liquid cooling maintains cells at 25±5°C, boosting capacity retention by 18–22% in EVs. Passive methods like phase-change materials (PCMs) suit solar storage. Insulated enclosures prevent -20°C capacity plunge in Arctic telecom stations. Thermal runaway thresholds (270°C for LiFePO4 vs. 150°C for NMC) allow simpler cooling systems but still require cell-level monitoring.
Cooling Method | Cost Increase | Capacity Benefit |
---|---|---|
Passive PCM | 8-12% | +15% @ 40°C |
Active Air | 15-18% | +22% @ 2C rate |
Liquid Cooling | 25-30% | +30% cycle life |
Advanced thermal management systems now incorporate predictive algorithms that anticipate load spikes. For instance, electric ferry operators using AI-driven cooling report 9% fewer capacity derating events during peak demand. Hybrid systems combining graphite heat spreaders with variable-speed fans demonstrate 28% better energy efficiency in data center backup applications compared to traditional approaches.
Why Do Application-Specific Load Profiles Dictate Capacity Design?
Peak vs. average loads determine C-rate requirements. Telecom towers need 2–4C pulses for RF amplifiers, while RVs prioritize 0.2C sustained draws. High C-rate demands reduce effective capacity due to Peukert’s effect—a 100Ah battery at 0.5C delivers ~95Ah, but at 2C, only 75Ah. Load cyclicity also affects BMS configuration for state-of-charge (SoC) balancing.
Expert Views
“LiFePO4’s capacity stability under dynamic loads is revolutionizing microgrid design,” says Dr. Elena Torres, Redway’s Chief Engineer. “We’re seeing 40% fewer oversizing demands compared to lead-acid in 5G base stations. However, integrators must recalibrate BMS algorithms monthly to account for lithium’s linear aging—a stark contrast to lead’s exponential decay. Our 2025 trials show AI-predictive balancing extends usable capacity by 22%.”
Conclusion
Designing LiFePO4 systems requires balancing application-specific energy needs with environmental, safety, and longevity constraints. Emerging technologies like modular architectures and smart BMS will enable finer capacity optimization, while standards reshape commercial viability. Always derate theoretical capacity by 15–30% for real-world variables.
FAQs
- How long do LiFePO4 batteries last in solar applications?
- Properly sized LiFePO4 solar systems achieve 8–12 years at 80% DoD, outperforming lead-acid by 3–5x. Capacity fade averages 2%/year vs. 4–6% for NMC.
- Can I increase LiFePO4 capacity after installation?
- Yes—modular systems allow capacity expansion via parallel module additions. However, new cells must be within 5% SoC of existing ones to prevent imbalance. Active balancers recommended beyond 3 modules.
- Are LiFePO4 batteries safe for home use?
- When properly enclosed and ventilated, LiFePO4 poses minimal fire risk—0.002% failure rate vs. 0.03% for NMC. Use UL-certified packs with thermal cutoff switches.